Lead
Chirality, derived from the Greek word “cheir” meaning hand, is a fundamental concept in chemistry that describes an object’s property of being non-superimposable on its mirror image. This property is not just an abstract mathematical idea but has profound implications in various scientific fields, particularly chemistry and biology. Understanding chirality is essential for comprehending molecular interactions and their impacts on our daily lives. This first of this blog series delves into the definition, historical context, significance in chemistry, everyday implications, and current research of chirality.
Chirality
Chirality refers to a geometric property of a figure or a molecule that makes it distinct from its mirror image. This is best illustrated by our hands: despite being mirror images, the left hand cannot be superimposed on the right hand. In the realm of chemistry, a molecule is considered chiral if it lacks an internal plane of symmetry and has a non-superimposable mirror image.
A common feature of chiral molecules is the presence of an asymmetric carbon atom, known as a stereocenter, which is bonded to four different substituents. These configurations lead to two non-superimposable structures called enantiomers. They are also referred to by chemists as chiral twins or handed molecules. Enantiomers exhibit identical physical properties in a non-chiral environment but differ in their interaction with other chiral entities, including biological systems and chiral reagents.
The distinction between chiral and achiral objects is crucial. Achiral objects are superimposable on their mirror images and often possess a plane of symmetry. In contrast, chiral objects do not possess such symmetry and therefore exist as distinct left- and right-handed forms.
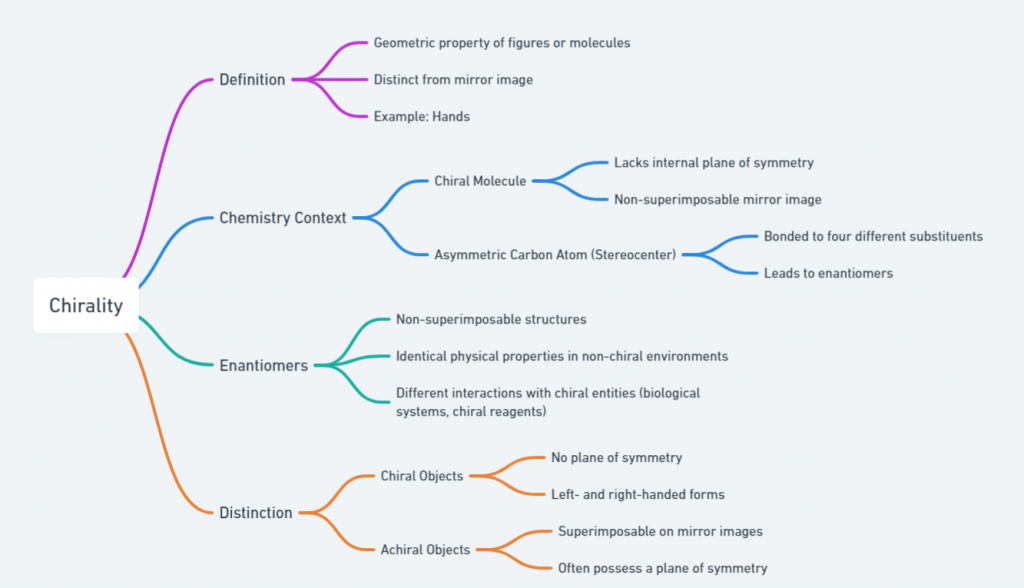
Historical Context and discovery
The concept of chirality in chemistry has its roots in the early 19th century. The first hints of chirality were observed through the study of the optical activity of certain substances. Jean-Baptiste Biot, a French physicist, discovered in 1815 that some organic compounds could rotate the plane of polarized light. This phenomenon, known as optical activity, suggested an underlying structural asymmetry in the molecules of these substances.

The breakthrough in understanding chirality came with Louis Pasteur’s work in 1848. Pasteur, a French chemist and microbiologist, was studying the crystalline forms of tartaric acid salts. He noticed that sodium ammonium tartrate crystals could exist in two forms that were mirror images of each other. By manually separating these crystals and dissolving them in water, Pasteur observed that each solution rotated polarized light in opposite directions. This led him to conclude that the molecules themselves were asymmetric.
Pasteur’s discovery laid the foundation for the field of stereochemistry, the study of the spatial arrangement of atoms in molecules. His work demonstrated that molecular asymmetry could directly influence physical properties such as optical activity, paving the way for future research into the implications of chirality in chemistry and biology. Chirality timeline can be accessed @ https://en.wikipedia.org/wiki/Chirality_timeline.
Chirality in Chemistry
Chirality is a cornerstone of modern chemistry, particularly in the study of stereochemistry. Enantiomers, the two mirror-image forms of a chiral molecule, are of significant interest because they can exhibit markedly different behaviors in chiral environments. These environments include biological systems, where the interaction between molecules and chiral biological structures can lead to different physiological effects.
Enantiomers and Diastereomers
Enantiomers are a specific type of stereoisomer, where each is a non-superimposable mirror image of the other. Despite having identical physical properties such as melting points, boiling points, and solubilities in achiral environments, enantiomers can differ dramatically in their biological activity. This difference is due to their interaction with other chiral molecules, such as enzymes and receptors in the body. In addition to enantiomers, there are diastereomers, which are stereoisomers that are not mirror images of each other. Diastereomers typically have different physical and chemical properties and can be separated by conventional methods such as distillation or chromatography.
Stereochemical Vocabulary
Before moving further, it is appropriate to introduce the stereochemical nomenclature system in place to enable you to follow when it is being employed at a later stage in the blog.
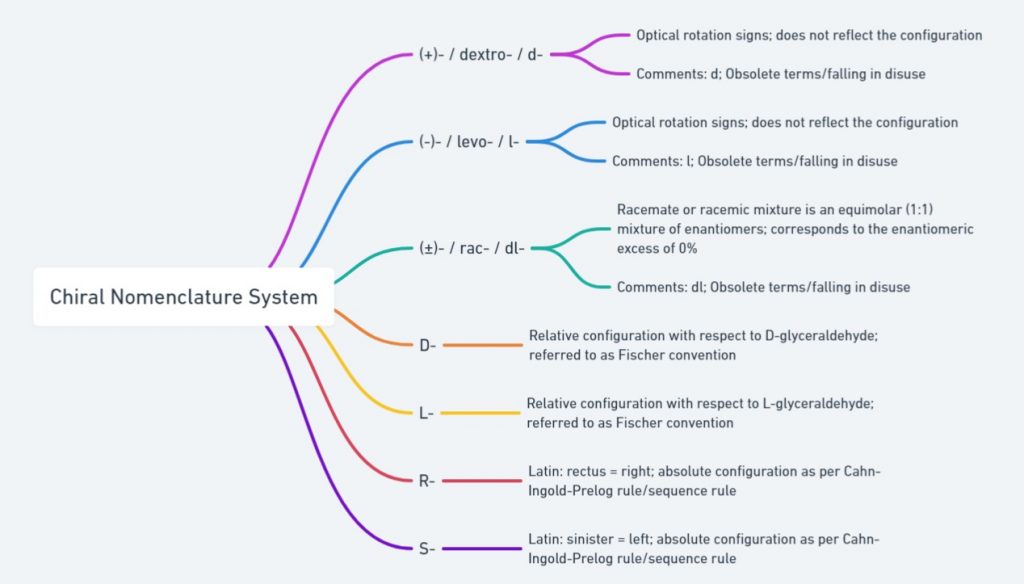
Naming Enantiomers
Cahn-Ingold-Prelog (CIP) system or R-/S-convention
Naming of enantiomers is important to understand ‘which structure refers to which enantiomer?’. The chirality of organic molecules is described by the Cahn-Ingold-Prelog (CIP) system. This system is also referred to as the R/S convention or Sequence rule. According to this system enantiomers are classified as ‘R’ (right from the Latin word ‘rectus’) and ‘S’ (left from the Latin word ‘Sinister’) absolute configuration based on the spatial orientation around the chiral center.
D-/L-system or Fischer convention
The Fischer convention was introduced to specify the relative configuration of a stereogenic center and uses the symbols D and L (capital letters). In general the D/L system of nomenclature is superseded by the Cahn-Ingold-Prelog (CIP) rule to describe the configuration of a stereogenic/chiral center. But D-/L-system of naming is still employed to designate the configuration of amino acids and sugars. So it is important to understand D-/L- system of naming enantiomers. To assign configuration as per Fischer convention the chiral molecule need to be represented using Fischer projection formula.
Chirality in Everyday Life
Chirality is not confined to the laboratory; it is a ubiquitous phenomenon in nature and has significant implications in everyday life. Many biological molecules, such as amino acids and sugars, are chiral. In living organisms, typically only one enantiomer of these molecules is active. For instance, all naturally occurring amino acids in proteins are of the L-configuration and are primarily used in protein synthesis, driving various biological functions. While the D-amino acids are commonly found in some bacterial cell walls and certain antibiotics. Similarly, sugars that go into the building up of nucleic acids are typically D-configuration. Read more @ <https://www.studysmarter.co.uk/explanations/chemistry/organic-chemistry/chirality/>.
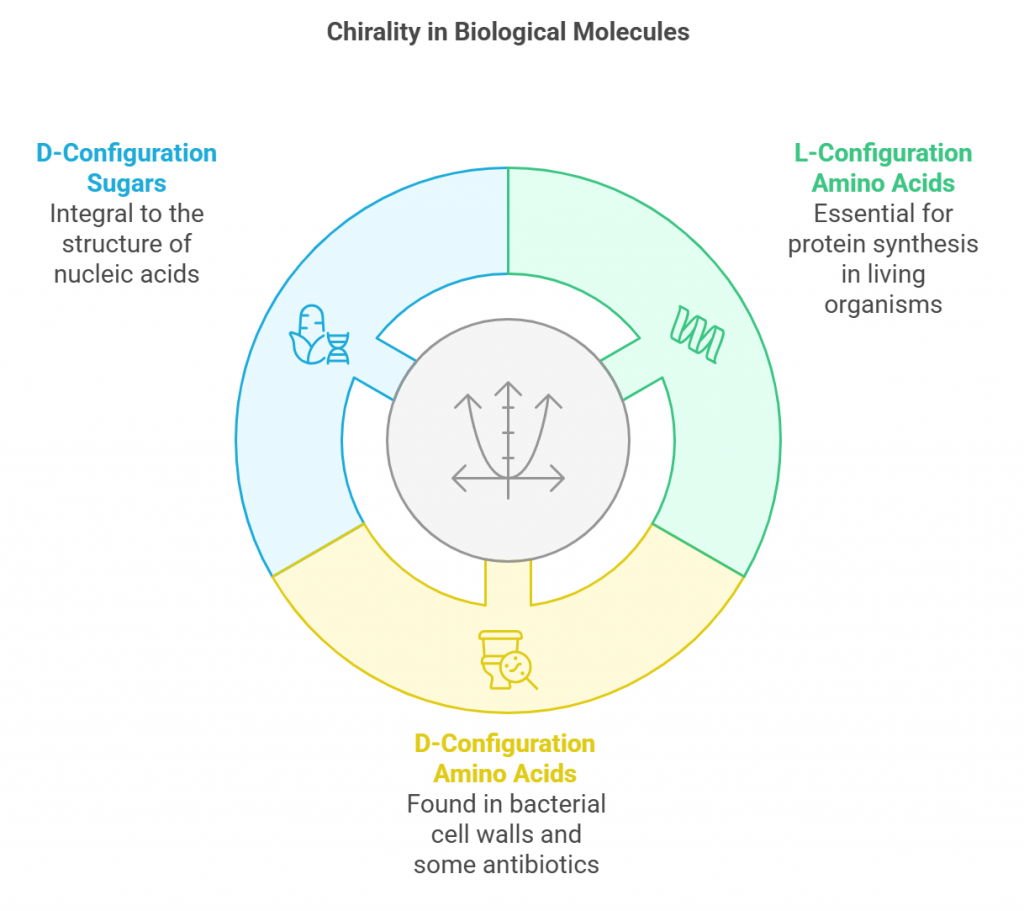
Chirality in Nature
In nature, chirality plays a crucial role in biological processes. Enzymes, which are chiral proteins, are highly specific catalysts that often interact only with substrates of a particular chirality. This specificity is essential for the proper functioning of biochemical pathways. The chirality of molecules can affect their taste and smell as well. For example, the two enantiomers of limonene have distinct scents: one smells like oranges, and the other like lemons.
Chirality in Pharmaceuticals
One of the most critical applications of chirality is in the pharmaceutical industry. The biological activity of a drug can be highly dependent on its chirality. Enantiomers of a chiral drug can have different pharmacological effects, where one enantiomer may provide the desired therapeutic effect while the other could be inactive or even harmful.
The thalidomide tragedy in the late 1950s and early 1960s is a sobering example of the importance of chirality in drug design. Thalidomide, initially marketed as a sedative and treatment for morning sickness, was later found to cause severe birth defects. This adverse effect was due to one enantiomer of the drug, while the other enantiomer was effective and safe. This incident highlighted the necessity of developing enantiomerically pure drugs and spurred regulatory changes requiring the evaluation of each enantiomer separately.
Chirality in Food and Flavors
Chirality also affects the food and flavor industries. The two enantiomers of carvone, for example, provide distinctly different smells: one enantiomer smells like caraway seeds, while the other smells like spearmint. This is due to the interaction of the enantiomers with chiral olfactory receptors in the nose, demonstrating how chirality can influence sensory perceptions. [the Nose ….. Knows..]
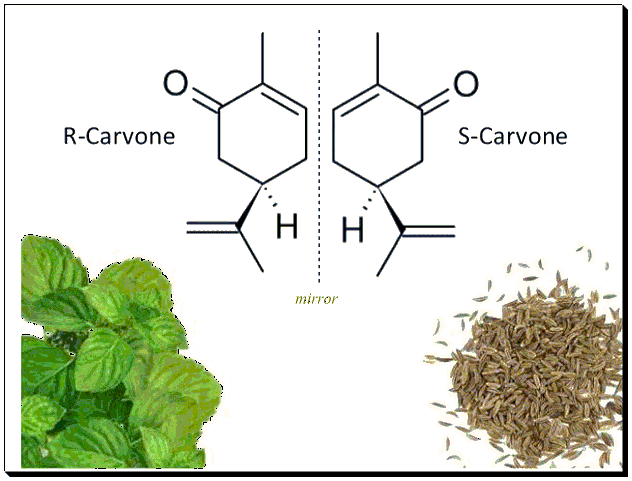
Chirality in Drug Design and Synthesis
Chiral synthesis (enantioselective synthesis, also called asymmetric synthesis) is the synthesis of a compound by a method that favors the formation of a specific enantiomer or diastereomer. It is a key process in modern chemistry and is particularly important in the field of medicinal chemistry, as the different enantiomers or diastereomers of a molecule often have different biological activities.
In the pharmaceutical industry, the role of chirality in drug design and synthesis is paramount. The therapeutic efficacy and safety of a drug can depend on its chirality. Developing enantiomerically pure drugs can enhance their effectiveness and reduce adverse effects. Advances in chiral synthesis and chiral separation techniques have enabled the production of single-enantiomer drugs, improving drug safety and efficacy.
The importance of chirality extends across various scientific disciplines and industries. In biological systems, chirality is fundamental to molecular recognition processes, such as enzyme-substrate interactions, receptor-ligand binding, and the replication of genetic material. The three-dimensional arrangement of atoms in chiral molecules determines their interaction with other chiral entities, which is crucial for the proper functioning of biochemical processes.
Chirality in Materials Science and Nanotechnology
Chirality also has significant applications in materials science and nanotechnology. Chiral molecules can be used to create materials with unique optical, electronic, and mechanical properties. For example, chiral liquid crystals are used in display technologies, such as liquid crystal displays (LCDs). In nanotechnology, chiral nanoparticles have potential applications in areas such as catalysis, drug delivery, and the development of novel materials. [Read more @ https://chiralpedia.com/chiral-materials.php].
Current Research and Future Directions
Research in chirality continues to advance, driven by the need for more precise and efficient methods of studying and utilizing chiral molecules. Recent developments include advanced spectroscopic techniques and computational models that allow scientists to predict and analyze the behavior of chiral compounds with greater accuracy.
Recent Advancements
Recent advancements in chiral analysis include the development of chiral chromatography techniques, such as chiral high-performance liquid chromatography (HPLC) and chiral gas chromatography (GC). These techniques enable the separation and analysis of enantiomers in complex mixtures, facilitating the study of chiral compounds in various fields.
Computational chemistry has also made significant contributions to the study of chirality. Computational models can predict the chiral properties of molecules, aiding in the design of chiral drugs and materials. These models can simulate the interactions of chiral molecules with biological targets, providing insights into their mechanism of action and potential side effects.
Emerging Technologies
Emerging technologies, such as 3D printing at the molecular level, offer exciting possibilities for creating custom chiral structures with specific functions. These technologies could revolutionize fields such as drug design, materials science, and nanotechnology, enabling the creation of tailored chiral compounds with precise properties.
Future Applications
Future research is likely to focus on the development of new materials and drugs that exploit chiral properties, as well as the exploration of chirality in previously unstudied systems. The potential for innovation in this field is vast, promising significant advancements in medicine, nanotechnology, and materials science. In medicine, the development of new chiral drugs could lead to more effective treatments with fewer side effects. In materials science, chiral materials could be used to create advanced technologies, such as sensors, catalysts, and optical devices. In nanotechnology, chiral nanoparticles could be used for targeted drug delivery, imaging, and the development of new materials with unique properties
Conclusion
Chirality is a fascinating and essential concept that influences many aspects of science and everyday life. From its historical discovery to its critical role in modern chemistry and biology, understanding chirality provides valuable insights into the molecular world. As research continues to uncover new applications and technologies, the importance of chirality is likely to grow, driving further innovation and discovery. Whether in the development of new drugs, the design of advanced materials, or the exploration of biological systems, chirality remains a cornerstone of scientific inquiry and progress.
Bibliography
Pasteur, L. (1848). “Researches on the Molecular Asymmetry of Natural Organic Products”. Comptes Rendus Hebdomadaires des Séances de l’Académie des Sciences, 26, 535-538.
Ghislaine Vantomme, Jeanne Crassous, Pasteur and chirality: A story of how serendipity favors the prepared minds, Chirality, 33, 10, 597-601, 2021. https://onlinelibrary.wiley.com/doi/full/10.1002/chir.23349
Eliel, E. L., Wilen, S. H., & Mander, L. N. (1994). Stereochemistry of Organic Compounds. Wiley-Interscience.
Roger A. Hegstrom and Dilip K. Kondepudi, The Handedness of the Universe, Scientific American, January, 108-115, 1990.
“Chirality timeline”, Wikipedia, Wikipedia Foundation, 24/05/2024. https://en.wikipedia.org/wiki/Chirality_timeline
Basic terminology of stereochemistry (IUPAC Recommendations 1996) G. P. Moss, https://www.degruyter.com/document/doi/10.1351/pac199668122193/html
K. Valliappan, Isomerism and isomeric drugs, Indian drugs, 33(3), 98-106, 1996 and references therein. https://chiralpedia.com/pdf/Indian-Drugs-Isomerism-and-Isomeric-Drugs.pdf
Noyori, R. (2002). “Asymmetric Catalysis: Science and Opportunities”. Angewandte Chemie International Edition, 41(12), 2008-2022.
Nguyen, L. A., He, H., & Pham-Huy, C. (2006). “Chiral Drugs: An Overview”. International Journal of Biomedical Science, 2(2), 85-100.
Jacques, J., Collet, A., & Wilen, S. H. (1981). Enantiomers, Racemates, and Resolutions. Wiley.
Berova, N., Nakanishi, K., & Woody, R. W. (2000). Circular Dichroism: Principles and Applications. Wiley-VCH.
Seeman, N. C., & Belcher, A. M. (2002). “Emulating Biology: Building Nanostructures from the Bottom Up”. Proceedings of the National Academy of Sciences, 99(Suppl 2), 6451-6455.
Mislow, K. (2002). “Molecular Chirality”. Topics in Stereochemistry, 6, 1-41.
Cintas, P. (2016). “Pasteur’s Chiral Molecules”. The Chemical Record, 16(6), 3008-3018.
Wolf, C. (2008). “Dynamic Stereochemistry of Chiral Compounds: Principles and Applications”. The Royal Society of Chemistry.
https://chiralpedia.com/blog/naming-enantiomers-the-r-s-system/
https://chiralpedia.com/blog/d-l-system-naming-the-left-or-right-hand-side/
https://chiralpedia.com/blog/fischer-projection-hassle-free-way-to-depict-a-stereoformula-in-2d-/
https://chiralpedia.com/blog/the-handed-world/“Chiral drugs”, Wikipedia, Wikipedia Foundation, 24/05/2024.
https://en.wikipedia.org/wiki/Chiral_drugs
https://chiralpedia.com/chiral-synthesis.php
https://chiralpedia.com/chiral-materials.php
“Chiral analysis”, Wikipedia, Wikimedia Foundation, 24/05/2024. https://en.wikipedia.org/wiki/Chiral_analysis
Adawy, A. Functional Chirality: From Small Molecules to Supramolecular Assemblies. Symmetry 2022, 14, 292. https://doi.org/10.3390/sym14020292
Other Blogs on #ChiralityonFriday
1. Introduction to Chirality: Understanding the Basics
2. Molecular Handedness: How Chirality Shapes Molecules
3. Chirality in Nature: From DNA to Snail Shells
4. The Chemistry of Taste and Smell: How Chirality Affects Senses
5. Chirality in Everyday Life: Hidden Handedness Around Us
7. https://chiralpedia.com/blog/chirality-in-materials-science-designing-with-handedness/
8. Chirality in Space: Cosmic Asymmetry and the Origins of Life
9. The Future of Chirality: Innovations and Emerging Trends
10. Chirality and You: Understanding and Appreciating Molecular Handedness