Introduction
Catalysis is a cornerstone of modern chemistry, playing a pivotal role in asymmetric synthesis. By facilitating the formation of chiral molecules with high enantioselectivity, catalytic asymmetric synthesis has revolutionized the production of pharmaceuticals, agrochemicals, and fine chemicals. The development of efficient chiral catalysts has enabled chemists to produce enantiomerically pure compounds more reliably and sustainably. This blog explores recent advances in catalytic asymmetric synthesis, highlighting innovations in chiral catalysts, notable reactions, and their industrial applications.
Asymmetric/Chiral Catalysts
Asymmetric catalysts, also known as chiral catalysts, are substances that can selectively promote chemical reactions in a way that produces a single enantiomer of a chiral molecule.
Chiral catalysis is presently the most efficient approach for achieving chiral synthesis. The search for new, effective chiral catalysts and ligands for enantiopure molecules is a continuous effort in the pharmaceutical, flavors and fragrances, and agrochemical industries. Approximately 85% of newly introduced pharmaceuticals exhibit chirality. This requirement has resulted in advancements in the field of asymmetric synthesis of chiral ligands and metal complexes.
Representative asymmetric catalytic systems include: Enzymes (Biocatalysts), Metal complexes, Small organic molecules, which are collectively referred to as three types of pillar-type asymmetric catalysts.
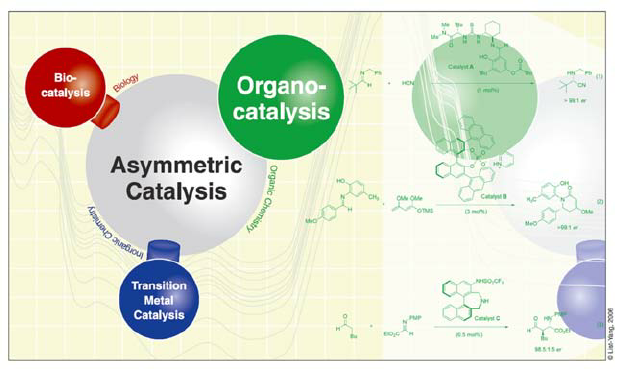
The three pillars of asymmetric catalysis: Biocatalyis, Metal Catalysis and Organocatalysis
Biocatalysts
Enzymes are nature’s chiral catalysts, and their application in synthetic chemistry has grown significantly. Advances in protein engineering and directed evolution have enabled the creation of enzymes with enhanced activity and enantioselectivity for non-natural substrates. These biocatalysts offer high specificity and can operate under environmentally benign conditions, making them attractive for industrial applications.
Although enzymatic methods have been used in fermentation for thousands of years, their molecular-level significance was first recognized in the early 1800s when scientists began studying yeasts for their fermentation capabilities. Initially, it was thought that the entire microorganism acted as the catalyst. However, the discovery of the first enzyme mixture “diastase” revolutionized the field by showing that specific parts of the organism were responsible for the reactions. This breakthrough ignited interest in enzymology, leading to key developments such as the lock and key model, cell-free fermentation, the understanding that enzymes are proteins, and the discovery of DNA structure. These milestones have laid the foundation for modern biocatalysis. The image below provides an overview.
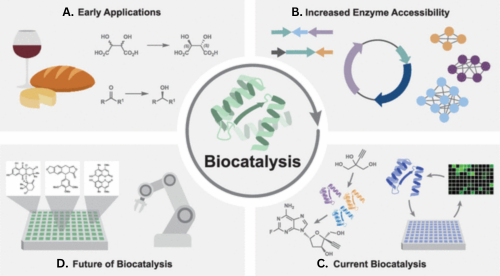
Biocatalysis has transcended early applications in fermentation to have an expanding footprint as an essential tool in the production of high value compounds.
A. Early Application: Early uses of enzyme-mediated transformations, such as fermentation, chiral resolutions, and functional group interconversions.
B. Increased Enzyme Accessibility: Recent advances in genome sequencing, gene synthesis, and bioinformatics increase the accessibility of obtaining enzymes.
C. Current Biocatalysis: Select strategies in modern biocatalysis include cascades, chemoenzymatic synthesis, and enzyme evolution.
D. Future of Biocatalysis: Application of computational methods for Biocatalysis.
Biocatalysis is widely acknowledged as a well-established technique for asymmetric synthesis, owing to the remarkable selectivity (enantio-, chemo-, and regio-) that enzymes frequently exhibit in various chemical reactions. The field has undergone significant advancement with the integration of emerging Molecular Biology techniques, which are utilized for the production and design of enzymes, along with the incorporation of process-development concepts (such as transitioning from batch to continuous reactors) and medium engineering strategies (such as non-aqueous media and immobilization). Biocatalysis can meet the requirements of contemporary organic synthesis, which necessitates a combination of high selectivity and efficiency, as well as sustainability and cost-effectiveness. Consequently, there has been a growing implementation of various industrial processes, which include a wide range of examples utilizing free enzymes, whole cells, systems biocatalysis, and multi-step processes.
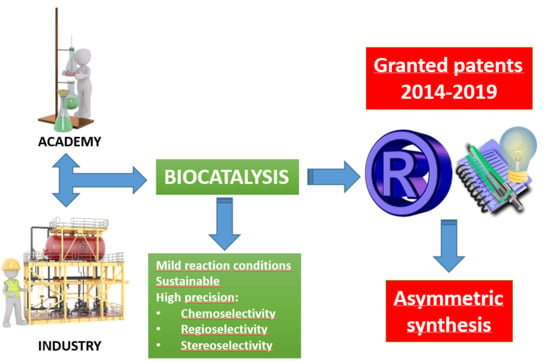
Below is a depiction of a patent survey conducted between 2014 and 2019, which provides more detailed information about the anticipated advancements in biocatalysis for asymmetric synthesis. The data accurately depict the contemporary trends in the field, sourced from academic and industrial groups, and encompassing various enzymes and chemical processes. Surprisingly, granted patents can offer additional evidence of the essential requirements of novelty, innovation, and resolution of industrial problems, which are necessary for intellectual property (IP) rights..
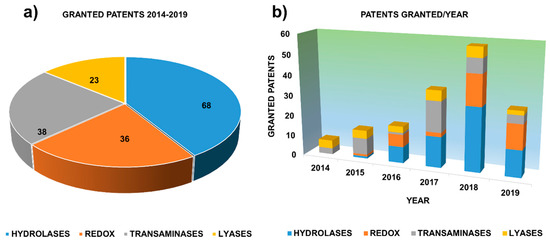
Patents granted in 2014–2019
Around 200 patents related to biocatalysis have been successfully granted within the last five years
Enzymes have been utilized for a diverse range of chemical processes for many years. Enzymes possess significant potency due to their ability to combine the benefits of a directing group that controls selectivity and a catalyst into a single reagent. Moreover, they can be employed alongside other enzymes in a one-pot reaction. In the last two decades, the integration of synthetic and enzymatic systems has facilitated numerous total synthesis projects. Moreover, enzymes are increasingly being employed as a standard practice in certain process chemistry teams within the industry. Previously, only a limited number of enzymes, such as lipases or ketoreductases (KREDs), were accessible for use in chemical synthesis. Nevertheless, the expansion of potential enzyme sources for applications in process chemistry has quickened, leading to a wide range of enzymes now accessible to researchers. The possibility of ‘bio-retrosynthesis’ was demonstrated in 2014 with the development of a complete enzymatic synthesis of the nucleoside didanosine. Retrosynthesis is a concept that involves breaking bonds in a target molecule to create simpler precursors using synthetic transformations. In the case of bio-retrosynthesis, an artificial enzyme cascade is designed as a synthetic biochemical pathway. This cascade utilizes enzymes as catalysts to achieve the necessary chemical reactions, providing a potential pathway to produce the desired target molecule.
Biocatalysis is facilitated by several factors, such as the design of the reaction, the selection and optimization of the biocatalyst, and the development of the bioprocess. It has been employed in the production of a diverse array of chemicals and pharmaceuticals, including the ones depicted in the Figure below.

Examples of different products synthesized using biocatalysis
For instance, nitrile hydratases are employed on a large scale, producing acrylamide in quantities of thousands of tons. Additionally, enzymes have been incorporated into detergents for over three decades. In recent times, there has been a growing prevalence in the utilization of proteins as catalysts in the chemical synthesis of intricate compounds, including pharmaceuticals. The synthesis of the HIV inhibitor islatravir, driven entirely by biocatalysts, showcases the effectiveness of integrating contemporary methods in designing novel enzyme cascades. These methods include repurposing established biosynthetic pathways, screening saturation mutagenesis libraries to identify enzyme variants, and employing directed evolution techniques to enhance enzyme stability and turnover.
Transition Metal Catalysts
Transition metal complexes are among the most versatile and widely used chiral catalysts in asymmetric synthesis. These complexes often feature a central metal atom coordinated to chiral ligands that create an asymmetric environment around the active site. This setup facilitates enantioselective reactions. Recent advances include the development of new chiral ligands that enhance the selectivity and efficiency of metal-catalyzed processes.
Knowles’s catalytic asymmetric hydrogenation
Similar to how the left hand is a reflection of the right hand, numerous molecules exist in two forms that have the same chemical composition but are mirror images of each other. The two forms can exhibit distinct characteristics and have contrasting impacts on the body. For instance, a particular variant of the limonene molecule emits an aroma reminiscent of oranges, while its reflection possesses a lemony scent with hints of turpentine.
An additional instance is the medication L-dopa. One variant, referred to as L, mitigates symptoms associated with Parkinson’s disease, such as tremors and rigidity. However, it is also available in an alternative form known as D-dopa, which possesses toxic properties. In the 1960s, the conventional procedure for producing L-dopa resulted in an equal quantity of both forms. The process of separating them after their creation proved to be both time-consuming and costly.
Dr. Knowles devised a method to optimize the manufacturing process in order to increase the production of the most sought-after variant of specific molecules, such as L-dopa. The tool he used was a catalyst, which is a substance frequently employed to accelerate a chemical reaction. He pioneered the technique of asymmetric hydrogenation, employing a catalyst to not only accelerate the reaction but also bias it towards the production of 97.5 percent L-dopa and only 2.5 percent of the undesired (D) form. Subsequently, Monsanto initiated extensive manufacturing of the medication, which continues to be a fundamental component in the treatment of Parkinson’s disease, particularly during its initial phases.
Knowles’ Pioneering Work and its Industrial application
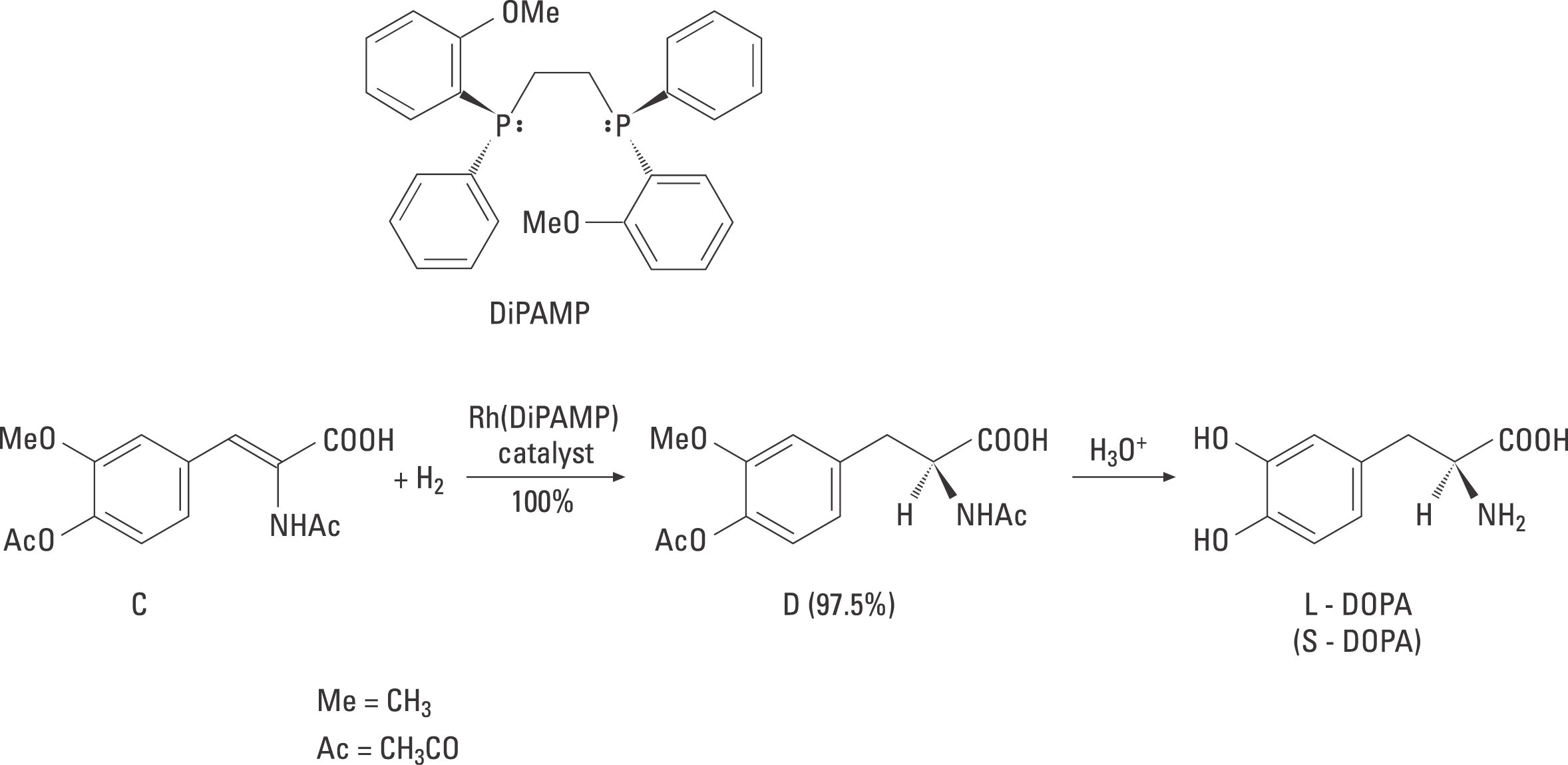
The first industrial catalytic asymmetric synthesis
Knowles and his colleagues utilized compound C as the initial substance in their industrial production of L-DOPA through synthesis. DiPAMP, one of the enantiomers, was utilized in the chiral hydrogenation process. Out of the product, the enantiomer D accounted for 97.5%. Subsequently, L-DOPA was obtained through the acid hydrolysis of D. Knowles’ objective was to create a method for producing the amino acid L-DOPA on an industrial scale, as it had demonstrated its effectiveness in treating Parkinson’s disease. Through experimentation with different structural variations of phosphines, Knowles and his colleagues efficiently generated effective catalysts that exhibited a high level of enantiomeric excess, specifically favoring L-DOPA. The ligand subsequently employed in Monsanto’s industrial synthesis of L-DOPA was the diphosphine ligand DiPAMP. A rhodium complex, when combined with the ligand shown in the image above, produced both enantiomers of DOPA in a 100% yield. The product consisted of 97.5% L-DOPA. Knowles quickly and successfully utilized his own fundamental research and the research of others to develop an industrial process for synthesizing a drug.
Noyori’s general hydrogenation catalysts
Ryoji Noyori, a Japanese scientist, has conducted extensive and intensive research and developed better and more general catalysts for hydrogenation. The implications of his research are highly significant.
In 1980, Noyori and his colleagues published an article illustrating the synthesis of both enantiomers of the diphosphine ligand BINAP, as shown in the Figure below. These catalysts, when combined with rhodium complexes, facilitate the production of specific amino acids with a chiral excess of up to 100%. Takasago International industry has been utilizing BINAP in the industrial synthesis of the chiral aroma compound menthol since the early 1980s.
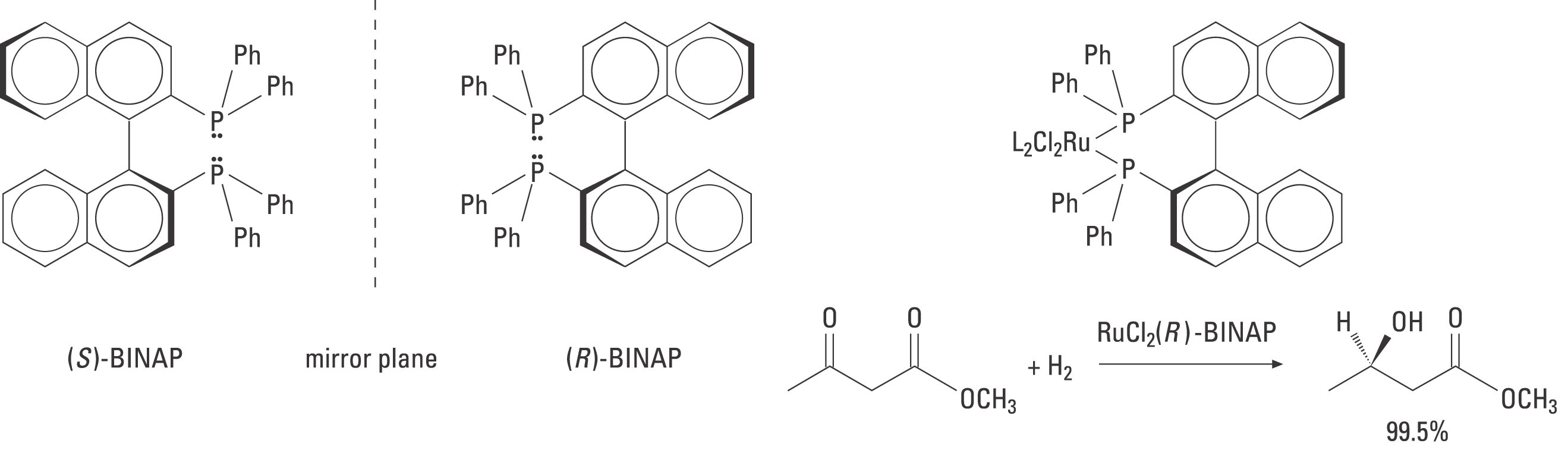
The two enantiomers of Noyori’s useful BINAP
Example of a stereoselective ketone reduction where the ester function is left intact. Noyori’s catalysts have found wide application in the synthesis of fine chemicals, pharmaceutical products and new, advanced materials.
Noyori saw the need for more general catalysts with broader applications. Exchanging rhodium, Rh(I), for another transition metal, ruthenium, Ru(II), proved, for example, to be successful. The ruthenium(II)-BINAP complex hydrogenates many types of molecules with other functional groups. These reactions give a high enantiomeric excess and high yields and can be scaled up for industrial use.
Noyori’s Work and its Industrial Application
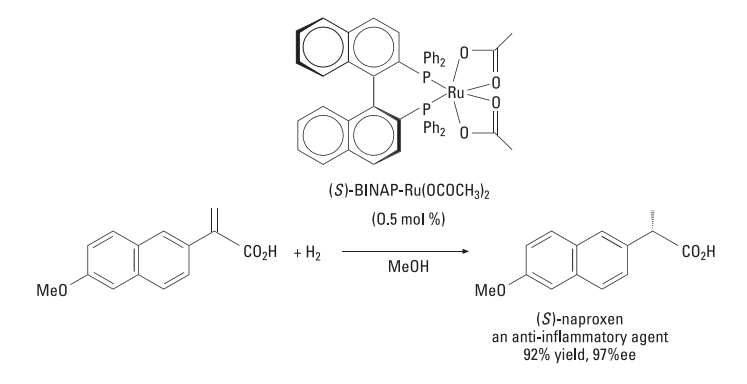
(S)-naproxen, anti-inflammatory agent, produced in high yield and ee (enantiomeric excess)
employing Noyori’s catalyst.
This is an illustration of a stereoselective reduction of a ketone, in which the ester group remains unchanged. Noyori’s catalysts have been extensively utilized in the synthesis of fine chemicals, pharmaceutical products, and novel advanced materials. Noyori recognized the necessity for more versatile catalysts that could be applied to a wider range of situations. The substitution of rhodium, Rh(I), with ruthenium, Ru(II), was found to be successful. The ruthenium(II)-BINAP complex is capable of catalyzing the hydrogenation of various molecules that contain different functional groups. These reactions exhibit a significant enantiomeric excess and high yields, making them suitable for industrial-scale production.
Sharpless’ chirally catalyzed oxidations
In addition to the progress made in chirally catalyzed hydrogenation reactions, Barry Sharpless has also developed chiral catalysts specifically designed for other significant reactions, such as oxidations. Hydrogenation eliminates a functional group by saturating the double bond, whereas oxidation results in an increase in functionality. This opens up new opportunities for constructing intricate molecules.
Sharpless recognized a significant demand for catalysts to facilitate asymmetric oxidations. His chiral epoxidation serves as an example of the several significant discoveries he has made. In 1980, he conducted successful experiments that resulted in the development of a practical technique for the catalytic asymmetric oxidation of allylic alcohols, leading to the production of chiral epoxides. This reaction employed the transition metal titanium (Ti) and chiral ligands, resulting in a high enantiomeric excess. Epoxides serve as valuable intermediate compounds in a wide range of synthesis processes. This approach has facilitated significant variations in structure and has been extensively utilized in both academic and industrial research. The chemical reaction for the production of the epoxide (R)-glycidol is illustrated below.
Sharpless’s Work and its Industrial Application
The emergence of the powerful Sharpless asymmetric epoxidation in the 1980s has stimulated major advances in both academic and industrial organic synthesis. Through the action of an enantiomerically pure titanium-tartrate complex, a myriad of achiral and
chiral allylic alcohols can be epoxidized with exceptional stereoselectivity. Interest in the Sharpless epoxidation as a tool for industrial organic synthesis increased substantially after Sharpless et al. had discovered that the asymmetric epoxidation process can be
conducted with catalytic amounts of the enantiomerically pure titanium-tartrate complex simply by adding molecular sieves to the epoxidation reaction mixture. Using this practical and reproducible catalytic variant, an industrial process for ton-scale productions of (S)- and (R)-glycidol and (S)- and (R)-methylglycidol has been developed. Many scientists have identified Sharpless’ epoxidation as the most important discovery in the field of synthesis
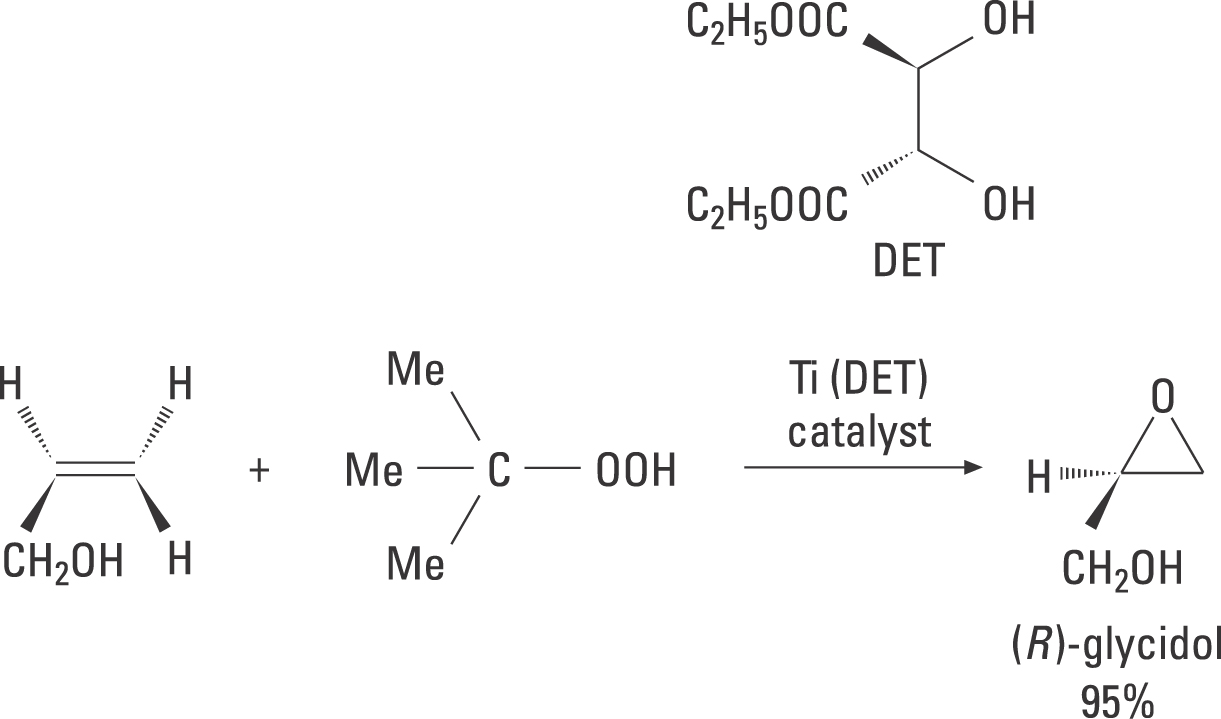
Glycidol is employed in the pharmaceutical sector for the synthesis of beta-blockers,
used as cardiovascular medications.
Sharpless’ epoxidation has been widely recognized by scientists as a pivotal breakthrough in the synthesis field. The allylic alcohol undergoes oxidation to form the epoxide (R)-glycidol, utilizing the oxidizing agent tertiary butylhydroperoxide in the presence of a catalyst. This catalyst is synthesized by combining titanium tetraisopropoxide with the diethyl ester of naturally occurring D-tartaric acid in the reaction mixture. The metal binds the chiral ligand, hyperperoxide, and substrate simultaneously, leading to the chiral epoxidation reaction.
Organocatalysts
Asymmetric organocatalysis, which involves using small chiral organic molecules as catalysts for stereoselective reactions, has become a crucial pillar in asymmetric catalysis, alongside enzymes and metal-based catalysts. This approach has found its way into the toolkits of scientists working on both academic and industrial projects. Over the past two decades, the field has introduced unique concepts and methods, earning recognition through prestigious awards, including the 2021 Nobel Prize in Chemistry awarded to Benjamin List and David MacMillan for their pioneering work in this area – “the development of asymmetric organocatalysis”. While the use of organic compounds as catalysts has been known for over a century, it was the groundbreaking publications by List, Barbas III, Lerner, and MacMillan in 2000 that sparked a new trend in organic synthesis. Thanks to MacMillan, the term “organocatalysis” has become widely accepted, leading to a significant increase in research groups focusing on this field.
It’s important to note that although the 2000 publications marked the beginning of modern organocatalysis and generated significant interest and competition in organic synthesis, there were several important contributions made earlier. These earlier works did not receive widespread attention at the time and were seen as isolated catalytic methods rather than foundational concepts for a broader field. Additionally, these earlier approaches were not classified as organocatalysis because the term did not exist then.
Development of Asymmetric Organocatalysis: Timeline
The story of asymmetric organocatalysis is truly amazing. The milestones in the historical developments are presented in the scheme below.

Selected milestones in the historical development of asymmetric organocatalysis.
The journey of asymmetric organocatalysis has been nothing short of remarkable. It all began over a century ago with the pioneering work of scientists like von Liebig, Knoevenagel, and Bredig, who demonstrated that small chiral organic molecules could catalyze asymmetric reactions. Fast forward to the latter half of the 20th century, and we saw the first highly enantioselective reactions being reported, showcasing the potential of this field. The real excitement, however, kicked off in 2000 with groundbreaking publications by MacMillan and List. Their work sparked a wave of interest and innovation in asymmetric organocatalysis, leading to rapid advancements and widespread recognition. This incredible journey reached a pinnacle in 2021 when Benjamin List and David MacMillan were awarded the Nobel Prize in Chemistry for their contributions to the development of asymmetric organocatalysis.
Benjamin List demonstrates unconventional thinking……
Several enzymes facilitate chemical reactions independently of metallic assistance. Conversely, the reactions are driven by a limited number of specific amino acids within the enzyme. Benjamin List posed a thought-provoking question: is it necessary for amino acids to be a component of an enzyme in order to facilitate a chemical reaction? Alternatively, could a solitary amino acid or other comparable simple molecules perform the same task?
…yielding a groundbreaking outcome
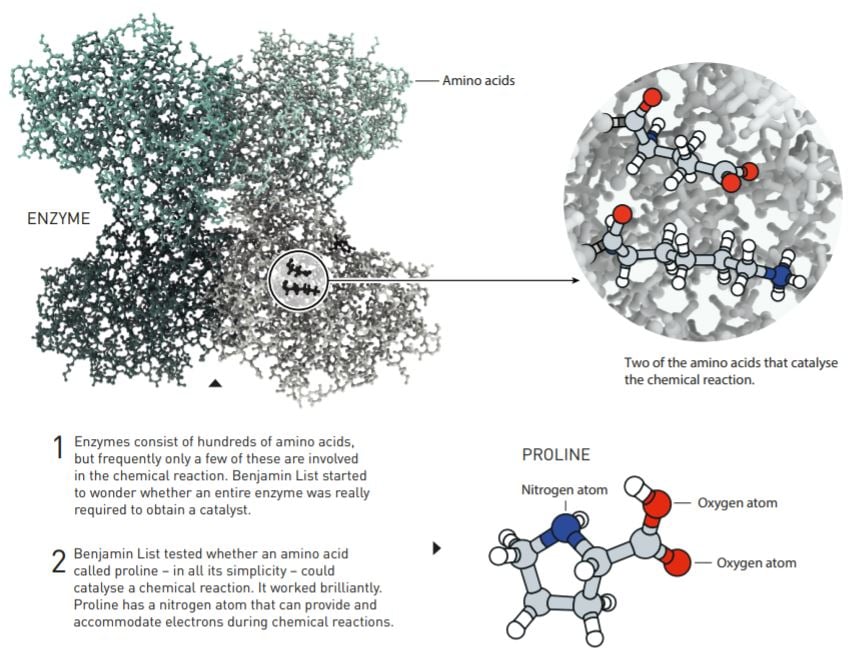
David MacMillan leaves sensitive metals behind…
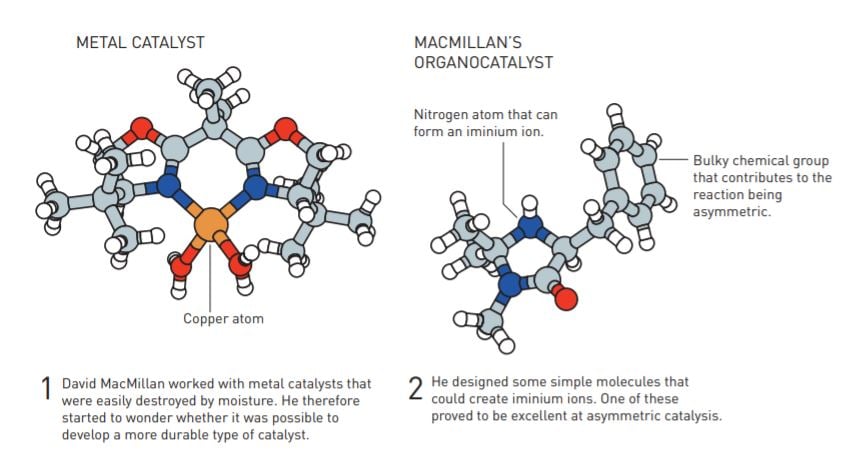
Chiral Molecular Catalysts: Design and Synthesis of Chiral Catalysts
Asymmetric Hydrogenation
Asymmetric hydrogenation is one of the most widely used catalytic processes in asymmetric synthesis. It involves the addition of hydrogen to prochiral substrates, resulting in the formation of chiral products. Recent advances include the development of more robust and selective hydrogenation catalysts that can operate under milder conditions, expanding the range of applicable substrates.
Noyori’s general hydrogenation catalysts – Chiral Molecular Catalysts: Beyond the Shape
Asymmetric catalysis involves chemical reactions that occur in four dimensions. Optimal efficiency can only be attained through the utilization of a synergistic blend of an ideal three-dimensional configuration (x, y, z) and appropriate kinetics (t). At present, the main approach to achieve efficient asymmetric catalysis involves employing a molecular catalyst composed of a metallic element and one or more chiral organic ligands.
The principle of asymmetric catalysis
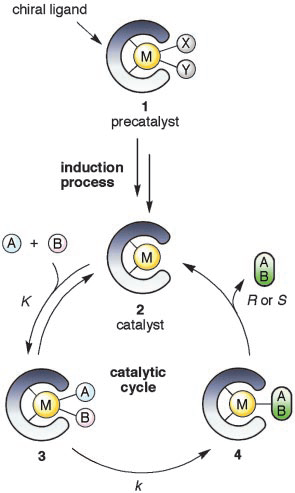
The principle of asymmetric catalysis with chiral organometallic molecular catalysts. M, metal; A and B, reactant and substrate; X or Y, neutral or anionic ligand.
The diagram on the right side depicts a typical (though not universally applicable) catalytic scheme. During the reaction, the initial precatalyst (1) undergoes a transformation to become the true catalyst (2) through an induction process. The true catalyst (2) then activates achiral molecules A and B, leading to the formation of the chiral product A-B in a catalytic cycle.
Asymmetric Allylic Substitution
Asymmetric allylic substitution (AAS) reactions involve the replacement of an allylic leaving group with a nucleophile in the presence of a chiral catalyst. These reactions are valuable for forming C-C and C-heteroatom bonds with high enantioselectivity. Recent progress includes the development of novel chiral ligands and transition metal complexes that improve the scope and efficiency of these reactions.
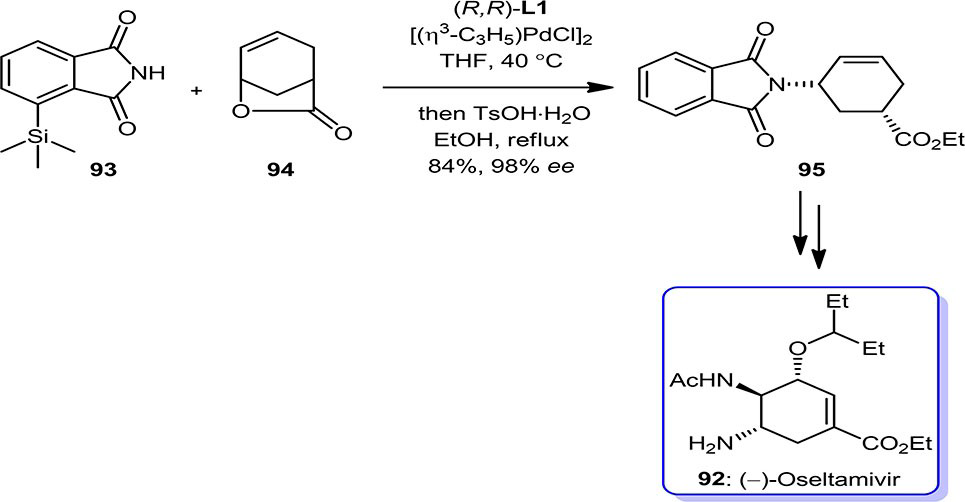
A novel Pd-catalyzed AAS was employed in the total synthesis of (-)-oseltamivir (92), anti-viral medication.
This method performed deracemizing lactone reaction with a nitrogen-centered nucleophile to open racemic cis-lactone (94) as the starting material and thus set an amino-group equivalent onto the ring enantioselectively. Dissimilar from the usual lactone-opening pathways, where nucleophiles commonly attack C7, the Pd-catalyzed AAS strategy leads in the nucleophilic addition at C2 or C4. Exceptionally for 94 bearing the tethered carboxylate as a leaving group, the essential carboxylic ester group can also be revealed by the Pd-AAS reaction. The total synthesis of the molecule ( )-92 was started with the commercially available racemic lactone (94), which was subjected to AAS using TMS-phthalimide (93) as the nucleophile in the presence of [(η3C3H5)PdCl]2 and the Trost ligand (R,R)-L1 in THF at 40°C to give the resulting TMS-carboxylate, which was then transformed into the ethyl ester with TsOH·H2O in refluxing ethanol in situ to yield the desired compound 95 in 84% yield and 98% ee in one pot. The conversion of (95) in several steps completed the synthesis of (-)-oseltamivir (92) as the expected natural product (Refer Scheme above)
Asymmetric C-C Bond Formation
Forming C-C bonds asymmetrically is a fundamental challenge in synthetic chemistry. Recent advances in this area include the use of new chiral catalysts that enable highly selective additions, cycloadditions, and cross-couplings. Innovations such as dual-catalysis, where two different catalytic systems work synergistically, have also been explored to enhance enantioselectivity and reaction efficiency.
Industrial Applications
Case Studies in Pharmaceutical Synthesis
Chiral drugs represent a significant portion of new pharmaceutical products. Catalytic asymmetric synthesis plays a crucial role in the production of these drugs. Recent case studies highlight the use of asymmetric hydrogenation and organocatalysis in the synthesis of active pharmaceutical ingredients (APIs) with high enantiomeric purity, ensuring their efficacy and safety.
Syntheses of (−)-paroxetine via organocatalysis

A novel approach, based on organocatalysis employing a chiral proline-derived catalyst led to a number of brief (formal) syntheses of paroxetine, an antianxiety-antidepressant agent.
The Michael reaction depicted in the above Scheme utilizes building blocks fluorocinnamaldehyde (70) and malonate derivative (71) as the starting materials, with silylated prolinol (XXVII) serving as the organocatalyst. This reaction, referred to as route (a), proceeds with high yields and stereoselectivity, resulting in the formation of the desired product (72). The entire process involves a three-step sequence. A previous study demonstrated the synthesis of (−)-paroxetine in six steps using organocatalyst (XVIII). This study also revealed the feasibility of a potentially scalable Michael addition reaction, above Scheme route (b).
Environmental and Economic Benefits
The use of catalytic asymmetric synthesis offers several environmental and economic benefits. By enabling the production of enantiomerically pure compounds, these methods reduce the need for extensive purification processes, lowering waste and energy consumption. Moreover, the development of more efficient catalysts reduces the amount of catalyst required, further decreasing costs and environmental impact.
Conclusion
The field of catalytic asymmetric synthesis has seen remarkable advancements in recent years, driven by innovations in chiral catalyst design and a deeper understanding of reaction mechanisms. These advances have expanded the scope of asymmetric synthesis, enabling the efficient production of chiral compounds for various industrial applications.

Asymmetric organocatalysis has developed spectacularly over the last decades, and its general importance was recently highlighted by the 2021 Nobel Prize (NP) in Chemistry.
Despite these successes, challenges remain, such as the need for even more selective and sustainable catalysts. Future research will likely focus on addressing these challenges, leveraging interdisciplinary approaches, and exploring new catalytic systems to continue pushing the boundaries of asymmetric synthesis. By staying abreast of these developments, chemists can continue to develop more efficient, sustainable, and versatile methods for the synthesis of chiral compounds, further enhancing the impact of asymmetric synthesis across multiple industries.
Further Reading
Corinna M. Reisinger, Subhas Chandra Pan, and Benjamin List, New Concepts for Catalysis, Systems Chemistry, 2008, http://www.beilstein-institut.de/Bozen2008/Proceedings/List/List.pdf. https://www.researchgate.net/publication/238658364.
Gavin, D. P., & Maguire, A. R. Chirality Related to Biocatalysis and Enzymes in Organic Synthesis. Chirality in Supramolecular Assemblies, 2016, 343–406. doi:10.1002/9781118867334.ch12.
de María, P.D.; de Gonzalo, G.; Alcántara, A.R. Biocatalysis as Useful Tool in Asymmetric Synthesis: An Assessment of Recently Granted Patents (2014–2019). Catalysts 2019, 9, 802. https://doi.org/10.3390/catal9100802.
Bell, E.L., Finnigan, W., France, S.P. et al. Biocatalysis. Nature Reviews Methods Primers, 2021, 1, 46. https://doi.org/10.1038/s43586-021-00044-z
Pyser, J. B., Chakrabarty, S., Romero, E. O., & Narayan, A. R. H. State-of-the-Art Biocatalysis. ACS Central Science, 2021, 7(7), 1105–1116. doi:10.1021/acscentsci.1c00273.
Rossino G, Robescu MS, Licastro E, et al. Biocatalysis: A smart and green tool for the preparation of chiral drugs. Chirality.
2022, 1‐16. doi:10.1002/chir.23498
Narmatha Senkuttuvan et al., The significance of chirality in contemporary drug discovery-a mini review. RSC Adv., 2024, 14, 33429-33448. DOI: 10.1039/D4RA05694A
https://www.nobelprize.org/prizes/chemistry/2001/summary
https://www.nobelprize.org/prizes/chemistry/2021/popular-information.
Ashna Garget al., Recent advances in catalytic asymmetric synthesis, Front. Chem., 2024, Sec. Organic Chemistry. https://doi.org/10.3389/fchem.2024.1398397
Ryoji Noyori*†, Masato Kitamura, and Takeshi Ohkuma, Toward efficient asymmetric hydrogenation: Architectural and functional engineering of chiral molecular catalysts, PNAS, 2004, 101 (15) 5356-5362. www.pnas.org/cgi/doi/10.1073/pnas.0307928100.
https://hwpi.harvard.edu/files/myers/files/18-noyori_asymmetric_hydrogenation_reaction.pdf
Mohammadkhani, L., & Heravi, M. M. Applications of Transition‐metal‐catalyzed Asymmetric Allylic Substitution in Total Synthesis of Natural Products: An Update. The Chemical Record, 2000. doi:10.1002/tcr.202000086.
Moschona, F.; Savvopoulou, I.; Tsitopoulou, M.; Tataraki, D.; Rassias, G. Epoxide Syntheses and Ring-Opening Reactions in Drug Development. Catalysts 2020, 10, 1117. https://doi.org/10.3390/catal10101117.
Ricci A. Asymmetric organocatalysis at the service of medicinal chemistry. ISRN Org Chem. 2014. doi: 10.1155/2014/531695. PMID: 24971178; PMCID: PMC4041019.
Olga García Mancheño, Mario Waser, Recent Developments and Trends in Asymmetric Organocatalysis, European Journal of Organic Chemistry, 2022, https://doi.org/10.1002/ejoc.202200950.
MacMillan, D. W. C. The advent and development of organocatalysis. Nature, 2008 455(7211), 304–308. doi:10.1038/nature07367.
Pranshu Vishwakarma and Abhishek Verma, A review on asymmetric organocatalysis historical works and present scenario, International Journal of Creative Research Thoughts, 2024, 12(5):2320-2882, DOI:10.1729/Journal.39332.
Biswa Mohan Sahoo and Bimal Krishna Banik, Organocatalysis: Trends of Drug Synthesis in Medicinal Chemistry, Current Organocatalysis, 2019, 6, 92-105. doi: 10.2174/2213337206666190405144423.
This article is quite detailed and captures a good review of the field including most recent developments much more than even ACS review papers, as it is written in a clear simple and illustrative style and facilitates a thorough understanding.
Appreciate your efforts in compiling this Sir.
Thank you very much for your kind words and appreciation. I’m delighted to hear that you found the article detailed and informative. It’s always my goal to present complex topics in a clear and accessible manner, and your feedback is truly encouraging. I’m glad the recent developments and illustrative style resonated with you. Your support motivates me to continue striving for excellence in my work.