Tagline:
“When scientific precision sharpened, so did regulatory vision -technology became the bridge between molecules and medicine.”
Introduction
The regulatory awakening around chiral drugs in the 1990s did not occur in isolation. It was powered, almost inevitably, by remarkable scientific and technological breakthroughs. Before sophisticated tools existed, distinguishing and isolating enantiomers was labor-intensive, error-prone, and prohibitively expensive. It was the advent of new chemical, analytical, and bioanalytical technologies that made the regulatory demands for stereochemical rigor feasible -and, ultimately, inevitable.
This episode explores how key scientific advances in asymmetric synthesis, chiral separation, enantioselective bioanalysis, and stereoselective pharmacokinetics reshaped the pharmaceutical industry’s relationship with chirality -and, in turn, catalyzed a profound transformation in regulatory science.
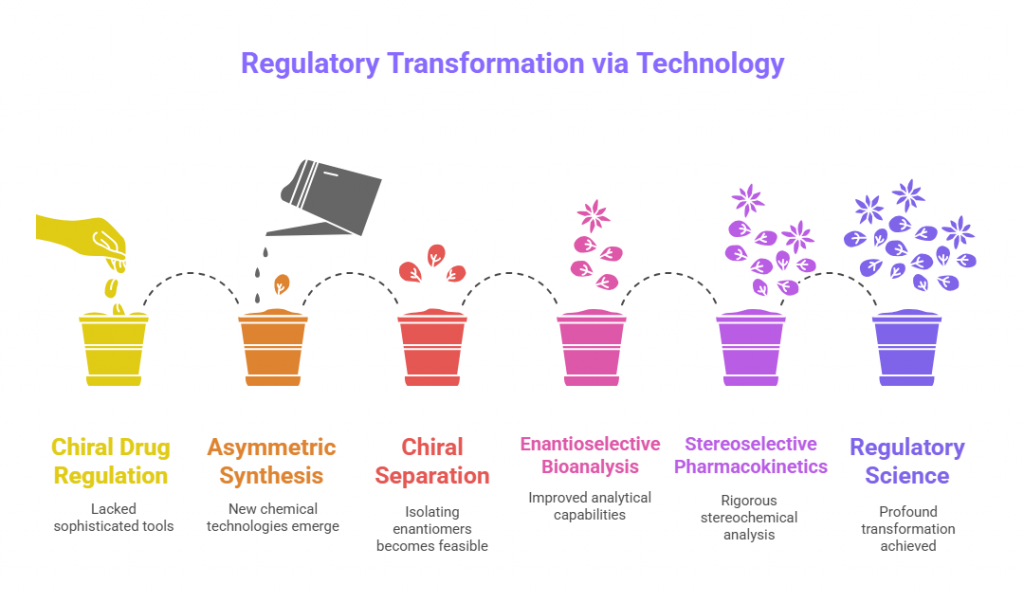
The Historical Limitations: Why Science Lagged Behind
In the pre-1990s world, the tools available for separating and analyzing enantiomers were extremely limited. Conventional chromatography methods could not distinguish between mirror-image molecules because enantiomers have identical physical and chemical properties in achiral environments (Wainer, 1993).
Early attempts at separating enantiomers relied on tedious and inefficient methods:
- Diastereomeric salt formation: converting enantiomers into diastereomers with chiral reagents, followed by physical separation.
- Repeated recrystallization: laborious and often incomplete.
- Classical resolution: highly specific to individual compounds and impractical at scale.
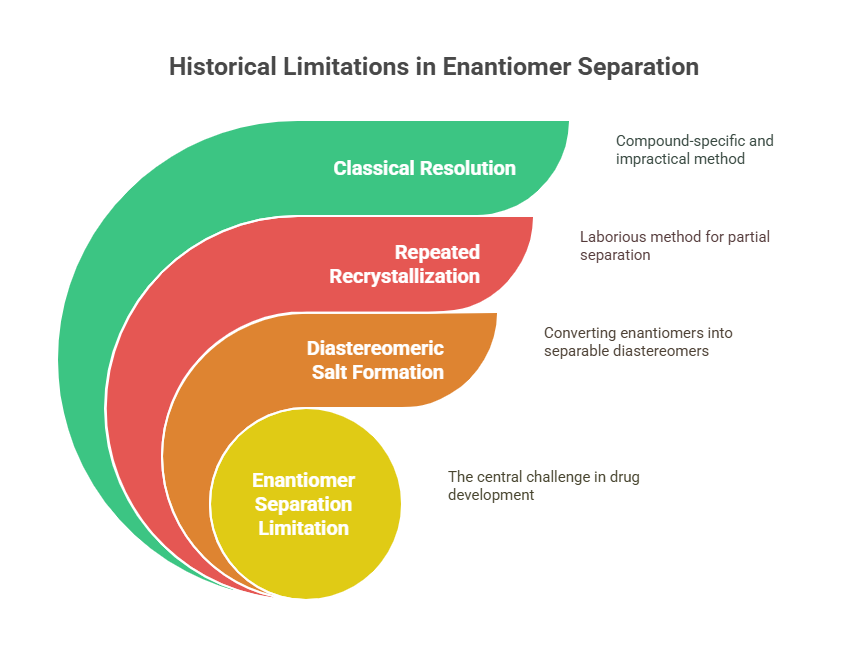
Because of these barriers, even when scientists knew that enantiomers could behave differently, they lacked the practical means to act on that knowledge during drug development.
Breakthrough 1: Advances in Asymmetric Synthesis
One of the first major technological shifts came from asymmetric synthesis -chemical reactions designed to preferentially produce one enantiomer over another.
Key Developments:
- Chiral catalysts: Sharpless asymmetric epoxidation, Noyori and Knowles asymmetric hydrogenation) allowed for the creation of single-enantiomer products directly from achiral precursors. Awarded Nobel Prize in Chemistry 2001
- Chiral auxiliaries provided temporary chiral environments during synthesis, facilitating selective reactions.
- Enzyme-catalyzed transformations offered environmentally friendly routes to chiral compounds (Faber, 2011).
- Organocatalysts: Benjamin List and David MacMillan received the Nobel Prize in Chemistry in 2021 for their individual contributions to the development of asymmetric organocatalysis, which involves using small chiral organic molecules as catalysts for stereoselective reactions, has become a crucial pillar in asymmetric catalysis, alongside enzymes and metal-based catalysts.
Regulatory Impact:
Asymmetric synthesis made it economically feasible to develop pure enantiomers as initial drug candidates, rather than treating racemates as unavoidable defaults. Regulators could now expect -and demand -enantiomerically pure development where scientifically justified.
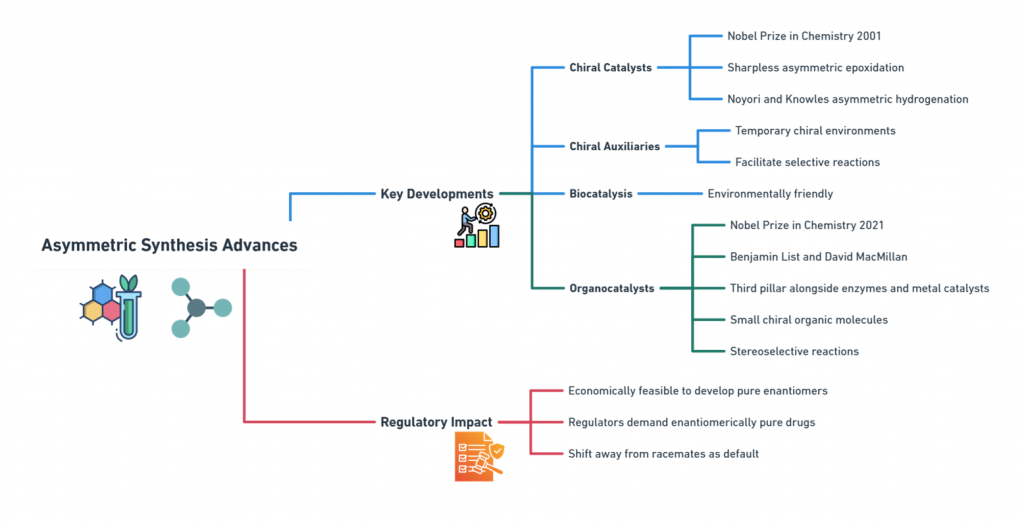
Breakthrough 2: Revolution in Chiral Chromatography
Perhaps the most critical enabling technology for regulatory evolution was the development of chiral chromatography.
Chiral Stationary Phases (CSPs):
- Protein-based CSPs (e.g., α₁-acid glycoprotein, bovine serum albumin) offered the first effective commercial tools for enantiomeric separation (Wainer, 1993).
- Polysaccharide derivatives (e.g., cellulose or amylose esters) expanded applicability to a broader range of compounds.
- Cyclodextrin-based phases enabled separations in aqueous environments.
Today, companies like Chiral Technologies and Daicel provide hundreds of CSPs optimized for different drug classes.
Other Techniques:
- Supercritical Fluid Chromatography (SFC) accelerated chiral separation, offering faster and greener alternatives to traditional HPLC (Lesellier, 2008).
- Capillary Electrophoresis with Chiral Selectors provided an additional orthogonal method for small molecules.
Regulatory Impact:
Chiral chromatography became the gold standard for quantifying enantiomeric purity in drug substances and drug products -a requirement embedded into ICH Q6A and enforced by FDA, EMA, and others (ICH, 1999).
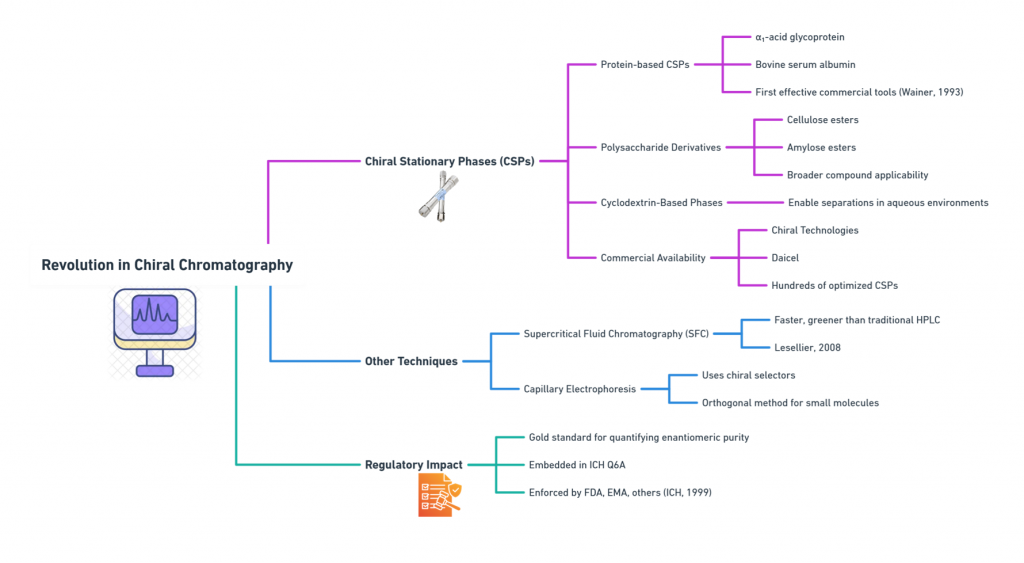
For regulators, the ability to insist on quantifying “the wrong enantiomer” was now a practical reality, not a theoretical aspiration.
Breakthrough 3: Enantioselective Bioanalysis and Pharmacokinetics
Regulatory policies also demanded pharmacokinetic profiling of individual enantiomers -but that required bioanalytical methods capable of distinguishing mirror images in complex biological matrices like blood or urine.
Advances:
- Chiral LC-MS/MS platforms integrated chiral chromatography with mass spectrometry for highly sensitive, enantioselective detection (Zhou et al., 2011).
- Enantioselective immunoassays were developed for specific cases where bioanalysis by antibody recognition was possible.
These tools enabled studies on:
- Differential absorption, distribution, metabolism, and excretion (ADME) of enantiomers.
- Stereoselective drug-drug interactions.
- In vivo racemization phenomena (e.g., thalidomide interconversion).
Regulatory Impact:
- The FDA’s 1992 policy statement explicitly required separate pharmacokinetic evaluation of enantiomers when feasible (FDA, 1992).
- EMA’s guidance echoed these demands, encouraging stereoselective bioanalysis in preclinical and clinical studies (EMA, 1994).
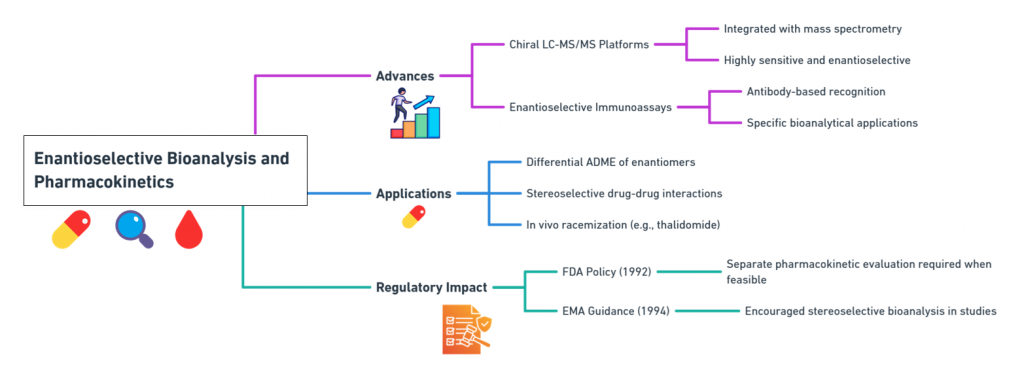
Without these technological advances, enforcing such policies would have been unrealistic.
Breakthrough 4: Understanding Stereoselective Metabolism
Beyond separation and detection, researchers gained new insights into how enantiomers could be metabolized differently.
Examples:
- Omeprazole, a proton-pump inhibitor, undergoes stereoselective metabolism leading to differences in exposure between the R- and S-forms (Andersson, 2001).
- Warfarin, a racemic anticoagulant, exhibits distinct metabolic pathways for each enantiomer, contributing to its narrow therapeutic index (Wadelius et al., 2007).
Understanding such differences became critical for:
- Dosing regimens.
- Risk assessments for drug-drug interactions.
- Justification for developing single-enantiomer formulations (e.g., esomeprazole).
Regulators began expecting sponsors to explore these issues during early-phase clinical trials, armed with new metabolic tools.
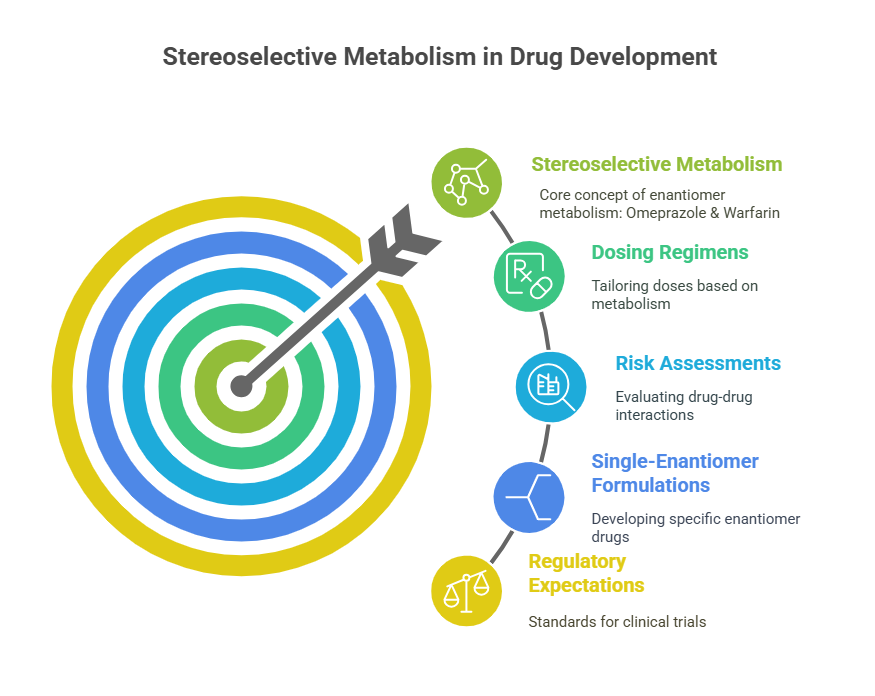
Integration into Regulatory Science: The New Normal
By the early 2000s, a feedback loop was established:
- Science enabled better stereochemical evaluation.
- Regulatory guidance demanded stereochemical data.
- Industry innovated further to meet these demands.
As a result:
- Chiral-specific data became standard in Module 3 and Module 5 of the Common Technical Document (CTD) for drug submissions.
- Enantioselective analytical validation was a regulatory expectation, not a luxury.
- Chiral impurity specifications became part of drug approval packages under ICH Q6A.
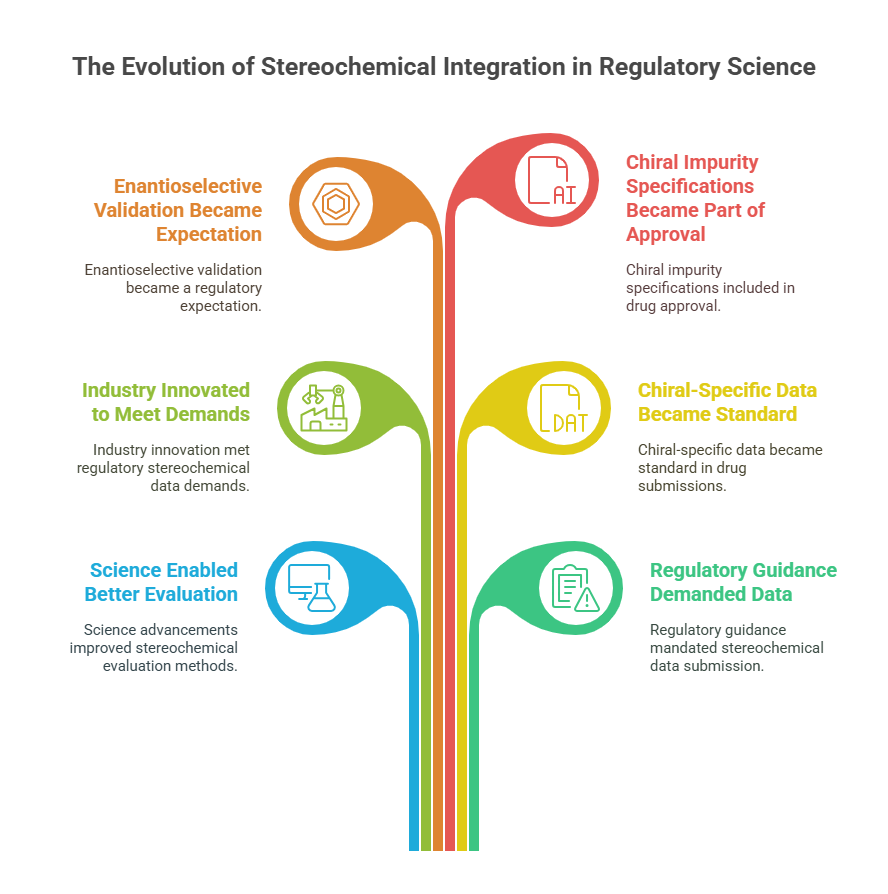
Key Case Studies: Science Meets Regulation
Esomeprazole (Nexium®)
- Developed as the S-enantiomer of omeprazole.
- Enabled through advances in chiral HPLC and stereoselective pharmacokinetics.
- Justified based on improved PK consistency and reduced interindividual variability (Andersson, 2001).
Levocetirizine (Xyzal®)
- Pure R-enantiomer of cetirizine.
- Developed using asymmetric synthesis and validated with chiral LC-MS/MS.
- Claimed to offer better efficacy-to-side-effect ratio (Simons, 2004).
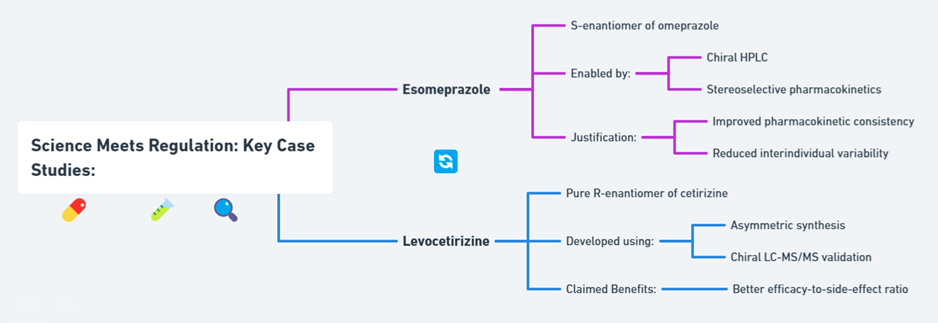
Both products leveraged scientific advances to meet -and benefit from -the evolving regulatory landscape.
Emerging Scientific Horizons
While 1990s–2000s technology made regulating traditional chiral drugs feasible, emerging fields are pushing the boundaries further:
- Atropisomers: molecules with axial chirality (e.g., certain kinase inhibitors).
- Chiral biologics: engineered stereocenters in peptide therapeutics.
- Dynamic kinetic resolution: on-the-fly resolution during metabolism.
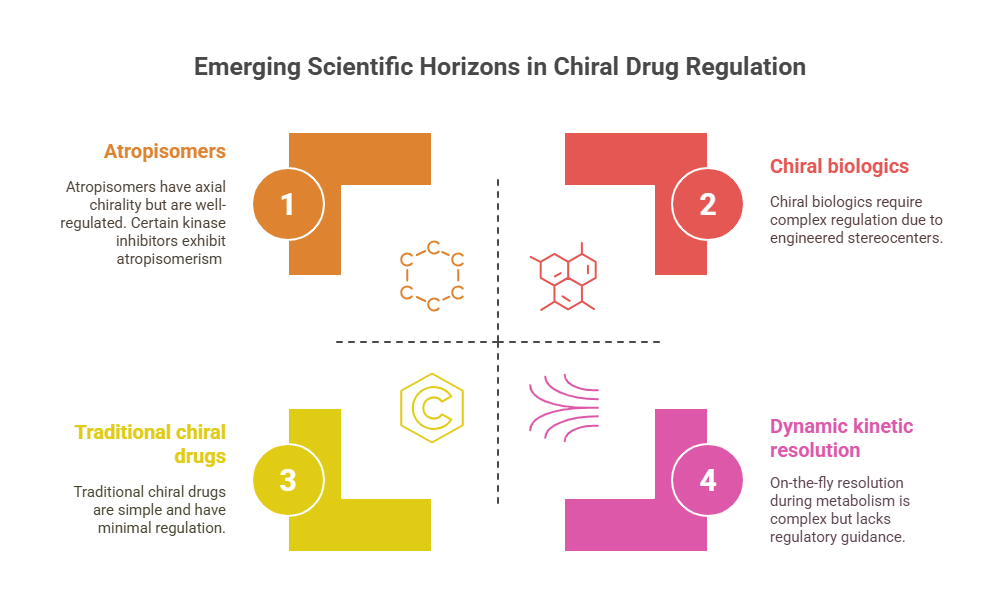
Future regulatory guidance will need to evolve further to accommodate these complexities -but the technological foundations are firmly in place.
Conclusion
Technological innovation was not merely an enabler but a driver of regulatory evolution around chiral drugs. Without the breakthroughs in asymmetric synthesis, chiral chromatography, bioanalysis, and metabolism studies, the regulatory frameworks introduced in the 1990s would have remained aspirational.
Today, the expectation that drug developers must rigorously characterize and control chirality is deeply ingrained -a testament to how technology and regulation co-evolve in the service of public health.
In the next episode, we will explore how regulators set detailed expectations for single-enantiomer development, building upon this scientific foundation.
What is in the next episode?
“Choosing the right mirror image is not just science -it’s policy. Join us in Episode 5: Choosing the Right Twin: Regulatory Expectations for Single Enantiomers.“
References
Andersson, T. (2001). Pharmacokinetics, metabolism and interactions of acid pump inhibitors: Focus on omeprazole, lansoprazole, pantoprazole, and rabeprazole. Clinical Pharmacokinetics, 40(7), 523–538.
European Medicines Agency (EMA). (1994). Investigation of Chiral Active Substances (CPMP Note for Guidance 3CC29a).
Faber, K. (2011). Biotransformations in Organic Chemistry (6th ed.). Springer.
Food and Drug Administration (FDA). (1992). Development of New Stereoisomeric Drugs (Policy Statement). Federal Register, 57(88), 22249-22250. https://www.fda.gov/regulatory-information/search-fda-guidance-documents/development-new-stereoisomeric-drugs
International Council for Harmonisation (ICH). (1999). Q6A: Specifications – Test Procedures and Acceptance Criteria for New Drug Substances and New Drug Products: Chemical Substances.
Knowles, W. S. (2001). Asymmetric hydrogenations. Angewandte Chemie International Edition, 40(2), 312–322.
Lesellier, E. (2008). The many faces of packed column supercritical fluid chromatography -A critical review. Journal of Chromatography A, 1217(11), 1560–1580.
Simons, F. E. (2004). Advances in H1-antihistamines. New England Journal of Medicine, 351(21), 2203–2217.
Wadelius, M., Chen, L. Y., Downes, K., Ghori, J., Hunt, S., Eriksson, N., … & Deloukas, P. (2007). Common VKORC1 and GGCX polymorphisms associated with warfarin dose. New England Journal of Medicine, 357(22), 2284–2293. DOI: 10.1038/sj.tpj.6500313
Wainer, I. W. (1993). The regulatory requirements for the separation, identification, quantitation, and pharmacological evaluation of the enantiomers of chiral drugs. Journal of Pharmaceutical and Biomedical Analysis, 11(6), 519–526.
Zhou, S. F., Xue, C. C., Yu, X. Q., Li, C., & Wang, G. (2011). Clinically important drug interactions involving mechanism-based inhibition of cytochrome P450 enzymes. Current Medicinal Chemistry, 18(30), 4986–5027. DOI: 10.1097/FTD.0b013e31815c16f5
Toenjes ST, Gustafson JL. Atropisomerism in medicinal chemistry: challenges and opportunities. Future Med Chem. 2018 Feb;10(4):409-422. DOI: 10.4155/fmc-2017-0152.